What is multi-messenger astronomy?
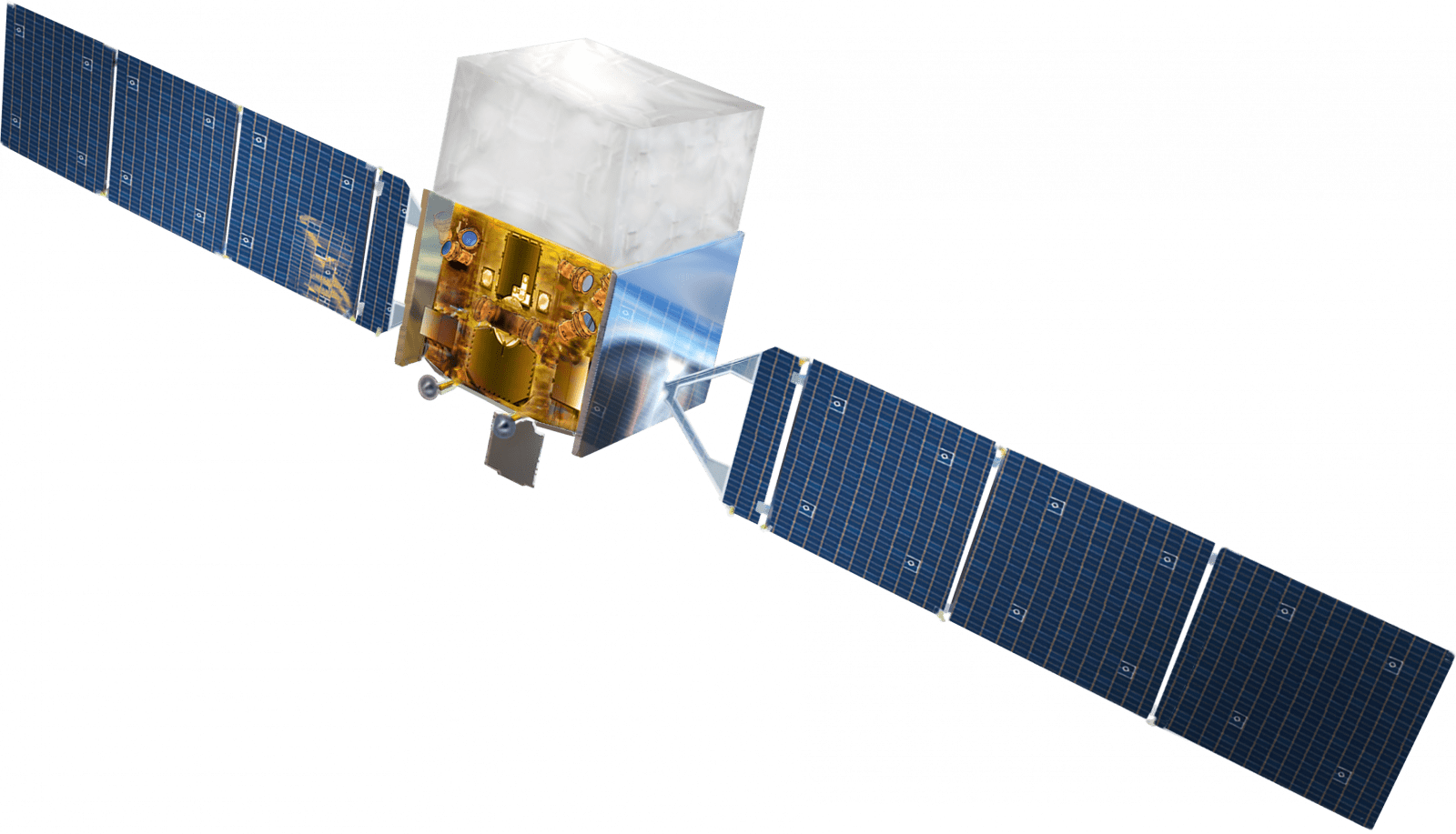
For thousands of years, astronomy was limited to looking up into the night sky with the naked eye. Early astronomers could use the stars to track the seasons and start tracing out the patterns of the moon, sun and planets.
Then the invention of the telescope during the Renaissance opened up entirely new avenues of inquiry. By the end of the 20th century, the entire electromagnetic spectrum — all energies of light — became available for scientists to study stars and galaxies.
The universe just talks to us in so many ways, and every time you find a new way of listening, you find something else.Ellen Zweibel, professor of astronomy
But astronomy now transcends light. Over the last several decades, astronomers and physicists have collaborated to gather information from several different sources, or “messengers” — including but extending beyond light alone to things like neutrinos and gravity waves — to study the cosmos. Multi-messenger astronomy tracks high-energy particles pelting Earth, particles passing through space and ripples in the fabric of space-time to answer questions about some of the most extreme events in the universe.
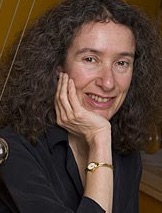
Zweibel
“The universe just talks to us in so many ways, and every time you find a new way of listening, you find something else,” says Ellen Zweibel, a professor of astronomy and physics at the University of Wisconsin–Madison who researches plasmas throughout the universe.
At first, we listened with light. And as each instrument capable of observing a new part of the spectrum — radio, or X-rays, or gamma rays — was turned on, new information flooded in.
But some of the universe’s most mysterious objects don’t give off any light to study. Black holes, for example, don’t allow any light to escape from their grasp. But their enormous gravitational presence can send waves through space-time that can be detected here on Earth, as was observed for the first time in 2015 during the merger of two black holes.
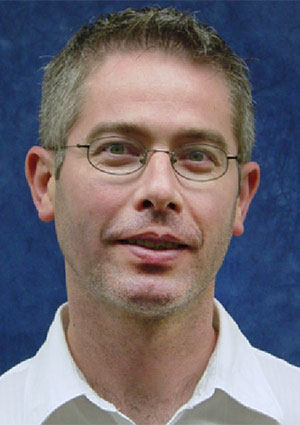
Brady
“It dawned on me: Holy cow, we just measured gravitational waves from a pair of black holes that collided out in the universe 1.3 billion years ago,” says Patrick Brady, a professor of physics at UW–Milwaukee and a member of the LIGO collaboration that detected the first gravitational waves in 2015, recalling the days following the discovery. “And for me it was just this beautiful moment that was a culmination of everything everyone in the collaboration had been working toward — just a beautiful feeling that we finally understood something new about the universe.”
Predicted by Albert Einstein, gravitational waves eluded direct measurement for a century. Although extremely powerful at their source, by the time these ripples in space-time arrive at Earth, they are minuscule. The 2015 gravitational waves changed the length of a four-kilometer measuring arm by a tiny fraction of the width of a proton.
Other messengers reveal information about an energetic universe but refuse to divulge their origin. Cosmic rays are a collection of high-energy particles constantly pelting the Earth from outside the solar system. They increase the radiation exposure of astronauts and can interfere with both spacefaring and terrestrial electronics.
“What accelerates cosmic rays to the energies that they have? The highest-energy cosmic rays that we’ve seen, it’s like a single proton with as much energy as a killer tennis ball (serve),” says Zweibel.
As they collide with our atmosphere, cosmic rays decay into a shower of other energetic particles. Some of these secondary particles can give off a shockwave of light as they pass through air, water or ice, giving astronomers information about the characteristics and direction of the original cosmic ray.
On a mountain peak in a national park in Mexico, 300 tanks holding 44,000 gallons of water apiece watch the sky. Light detectors inside the water tanks can collect the light produced by disintegrating cosmic rays. This international observatory, HAWC, is part of the Wisconsin IceCube Particle Astrophysics Center (WIPAC) at UW–Madison and is supported in part by the National Science Foundation (NSF). Its mission is to understand the origin of cosmic rays.
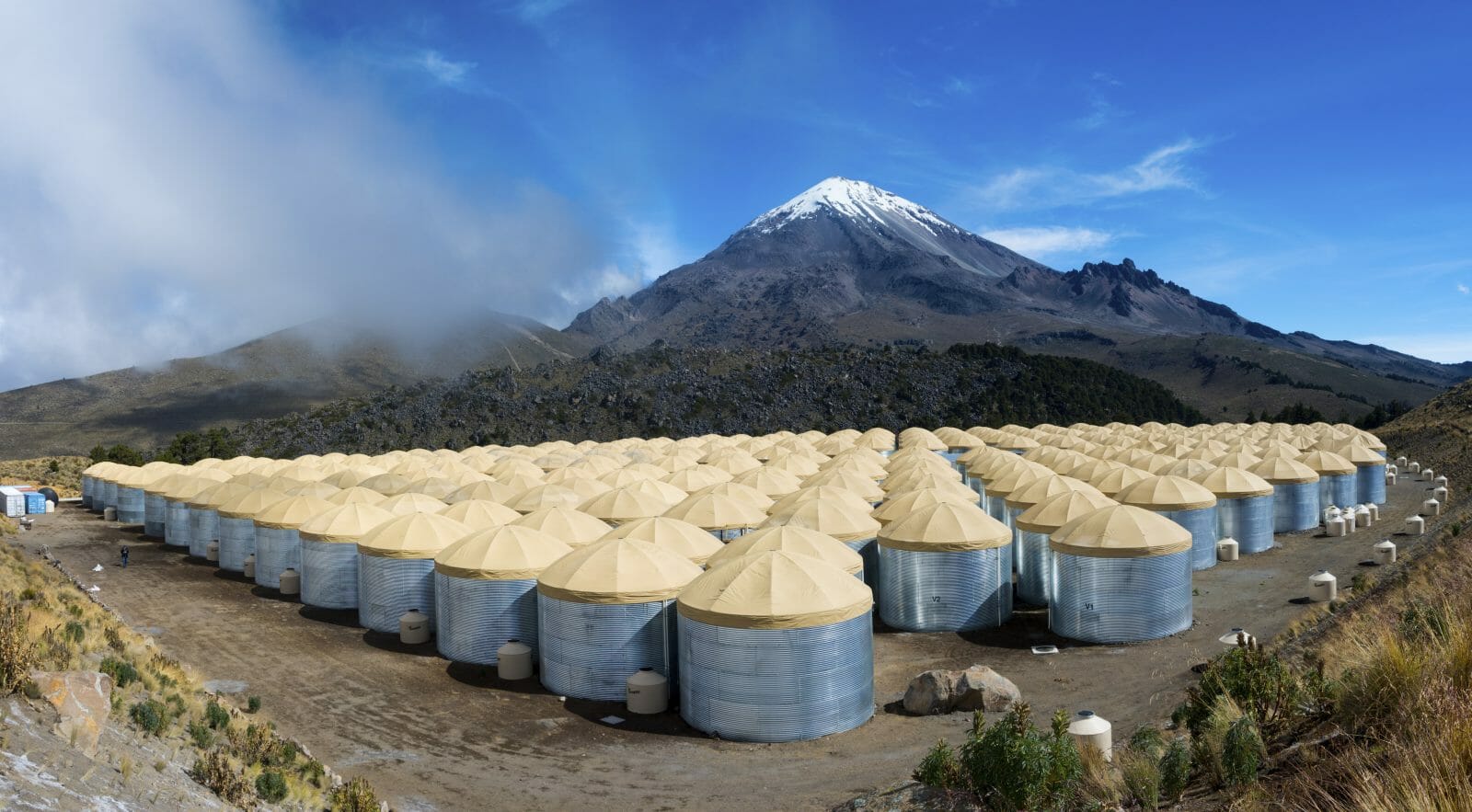
But because cosmic rays are charged particles, they are deflected by the magnetic fields that are pervasive in the universe as they travel to Earth. Although HAWC and other observatories can trace the direction of cosmic rays, their origins in the cosmos are obscured by this magnetic whiplash.
“But neutrinos don’t suffer from that problem,” says Zweibel.
Like cosmic rays, neutrinos are constantly streaming toward Earth — billions are passing through you every second. Importantly, neutrinos carry no charge, so their path to Earth, and thus their origin, is not obscured by magnetic fields. But neutrinos are loath to interact with most matter, only occasionally colliding with the nucleus of an atom and creating secondary particles that give off light like that of decaying cosmic rays.
The more mass you can put in their way, the more likely you are to catch a neutrino.
So astrophysicists are using a billion tons of ice at the South Pole to track these mysterious particles, which are created in extremely energetic cosmic events. Spread over a cubic kilometer of ice, more than 5,000 sensors watch for the light shockwave produced when neutrinos run into an atom of ice. This massive experiment, IceCube, is another WIPAC program, and identified the first high-energy neutrinos from outside our solar system and studied the lower-energy neutrinos coming from the sun. The observatory is run by an international collaboration, with NSF contributing substantially to the observatory’s construction and operation.
Light still has a vital role to play in multi-messenger astronomy. A gravitational wave event measured by LIGO in 2017 documented the merger of two neutron stars. Thanks to automated detection systems that alerted other, light-based observatories, those gravitational waves were correlated with bursts of gamma rays coming from the same source. This was the first proof of a longstanding idea that colliding neutron stars could emit gamma rays, and the observation helped astronomers understand how heavier elements like gold are formed in these violent events.
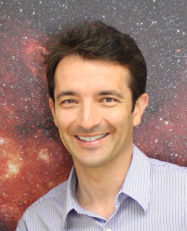
Heinz
Multi-messenger astronomy also increasingly relies on large collaborations. IceCube comprises 300 physicists, computer scientists and engineers. More than 1,000 scientists around the world contribute to LIGO. Such large teams have been the standard in particle physics for some time.
But astronomers interested in understanding the most extreme and mysterious objects in the universe are having to become more comfortable operating in large groups to get answers, says UW–Madison Professor of astronomy Sebastian Heinz.
“If you want to find something really small and difficult, you have to have a big team,” says Heinz.